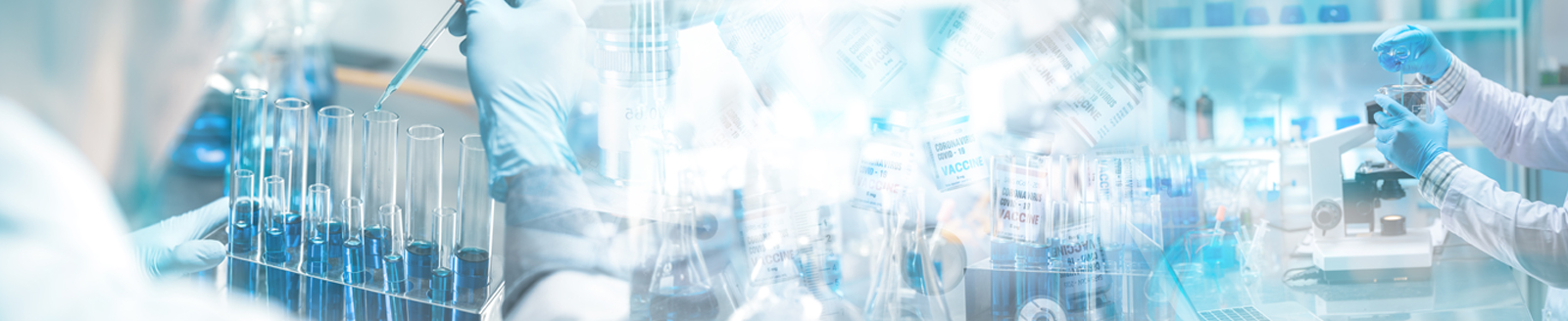
Science
Our Approach
VCCT Inc. is dedicated to the development and application of advanced cell therapy aimed at retinal degeneration diseases. Our prime objective is to make therapy available for every individual patient. To achieve this goal, we have developed unique techniques to specifically induce and manufacture retinal cells (photoreceptor and RPE) using iPS cells. We are also conducting research into the patient diagnosis, best surgical techniques and post-operative care to go along with the transplantation of therapeutic cells. We believe this holistic approach of optimizing an end-to-end therapy, encompassing patient selection, tailored treatment, and eventual long-term follow-up, is integral for to maximizing patients’ quality of life.
Outer Retinal Disorders
In societies undergoing significant aging, retinal degenerative disorders like Retinitis Pigmentosa (RP) and age-related macular degeneration (AMD) are becoming prominent causes of mid-life blindness. Although the specifics of these disorders may vary, they uniformly involve degeneration of photoreceptors and retinal pigment epithelium (RPE) cells located at the outer retina (hence collectively referred to as “outer retinal disorders”). Progression of these disorders is irreversible, and no effective treatments exist today. Therefore, there is an urgent demand for the development of a new, innovative treatment.
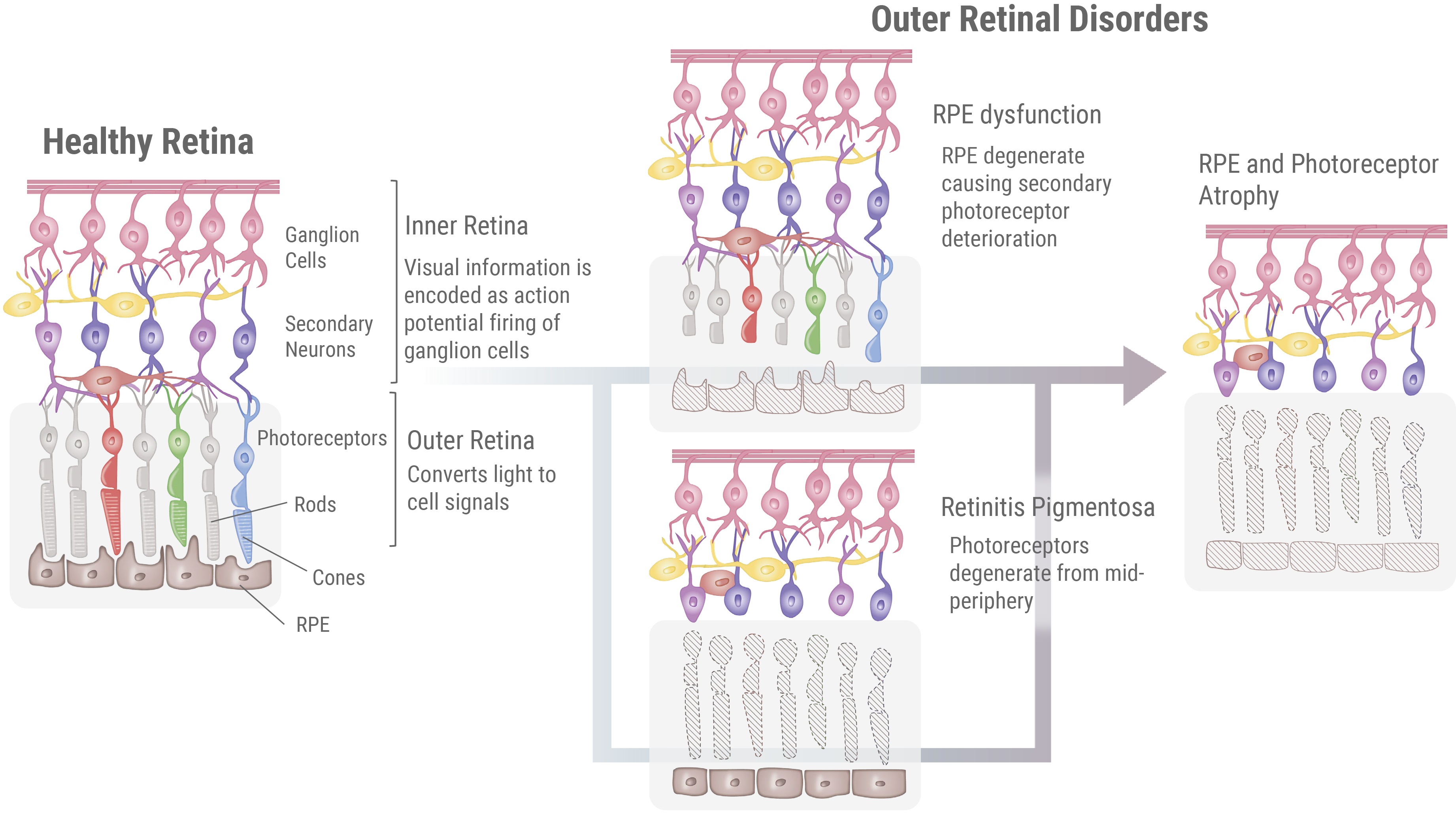
What is Retinitis Pigmentosa (RP)?
Retinitis Pigmentosa (RP) is a set of genetic disorders leading to the gradual degeneration of rod cells, the photoreceptors responsible for vision in dim light. As the condition worsens, it can start to affect cone cells, which manage central vision, color perception, and sharpness of vision, possibly culminating in complete blindness. Currently, there are more than 250 identified genes associated with RP, although the specific function of many of these genes is not yet fully understood. The pattern of inheritance can vary greatly depending on the causative gene, with all forms being reported: autosomal dominant, autosomal recessive, and X-linked. However, there are also isolated cases where no other instances of the condition can be traced within the patient’s family lineage. The onset age of Retinitis Pigmentosa can vary based on the causative gene and environmental factors is variable, but it typically manifests between the ages of 20 to 40, and symptoms gradually progress over a span of 5 to 10 years (though some individuals might exhibit symptoms as early as childhood or in their teens).
Current state of RP treatment and its limitation
In Japan, RP can be diagnosed successfully for 40-50% of cases via genetic testing. RP is a rare disease, affecting one in every 4,000 to 8,000 people. This translates to an estimated 30,000 patients in Japan alone, and close to 3 million people globally.
The first sign of this disease is usually night blindness, making it hard to see in dim light. This is typically followed by slow narrowing of vision, which starts from the outer visual field and moves inward. As the disease progresses further, it often results in reduced sharpness of vision and difficulties in distinguishing colors. The pace at which these symptoms progress can vary widely among patients, and the sequence in which they appear can also be different. For instance, some patients may first notice a decrease in their vision clarity or color discrimination abilities before they experience night blindness.
At present, there is no definitive cure for RP. Treatment options are typically symptomatic, including the use of sunglasses to reduce glare and the administration of Vitamin A or circulation-enhancing medications. Gene therapy and artificial retinas are beginning to be utilized, but as of now, these treatments are not widely available or accessible. We are developing regenerative medicine that aims to restore ocular functions.
What is Age-Related Macular Degeneration (AMD)?
Age-Related Macular Degeneration (AMD) is one of the most common causes of blindness worldwide, particularly among the elderly. The global prevalence is 8.7% leading to an estimate that about 288 million people in western countries will be afflicted by 2040. The early stages of AMD are characterized by features known as drusen and pigment loss from retinal pigment epithelium (RPE) cells. The progression of AMD is marked by the widespread degeneration of RPE cells and the formation of choroidal neovascularization (CNV). Advanced cases, directly linked to a decrease in quality of life (QOL) through central visual field impairment, are classified into two forms: atrophic and exudative. In exudative forms, CNV leads to blood and lipid leakage beneath the retina, and fibrous scarring, which cause the degeneration of visual cells and visual impairment. Presently, the available treatment includes intravitreal injections of anti-vascular endothelial growth factor (anti-VEGF) agents for the exudative type, and complement inhibitory drugs for the atrophic type. These treatments aim to curb disease progression but require regular, long-term interventions. However, neither treatment can restore visual function once the retina has already degenerated. Therefore, for the progressive cases of AMD, reconstructing the retina through cell transplantation remains the only means to preserve or restore visual function.
What is “RPE-impaired disease”
RPE plays vital roles in the maintenance of our vision. However, its normal function can be perturbed by genetic abnormalities or aging that results in the accumulation of oxidative stress and waste. This RPE dysfunction is the primary cause for a variety of retinal diseases, which are collectively referred to as “RPE-impaired disease”[Reference 1]. The RPE-impaired diseases include the aforementioned Retinitis Pigmentosa with genetic mutations affecting RPE genes (RPE65, MERTK, RDH5, etc.) as well as AMD, which has been the target of our clinical trials. Furthermore, diseases like crystalline retinopathy, Best‘s disease, Stargardt disease, high myopia, multifocal retinal pigment epitheliopathy, and pigmentary striae also compose of the RPE-impaired disease. Currently, the total number of patients affected by this umbrella disease is not fully understood.
The Current State of Treatment and Its Limitations for RPE -impaired diseases
There is currently no definitive cure for the RPE-impaired disease. However, one therapy has recently gained regulatory approval – a gene therapy targeting RPE65 gene mutations associated with protein deficiency in RP. It was approved in the U.S in 2017, and in Japan in 2023. Though this treatment is promising, it‘s only effective when administered early in the disease progression. So, for patients with advanced stages of the disease, this therapy might not be applicable.
For patients with AMD, the current treatment involves the use of antioxidant supplements and anti-VEGF agents. Supplements including vitamins, lutein, and zeaxanthin are utilized to shield retinal cells from oxidative stress. Anti-VEGF agents, on the other hand, are used to treat exudative AMD. They work by blocking VEGF‘s interaction with its receptors, thereby preventing abnormal blood vessel growth. Despite these interventions, treatment ceccation can often lead to high rates of disease recurrence.
There has been some progress in treating dry AMD, with the recent approval of an anti-complement drug. This drug is anticipated to slow down the progression of Geographic Atrophy (GA), a condition associated with dry AMD. However, it’s not capable of reversing already lost retinal cells. Due to this lack of definitive cure, RPE regenerative therapy, with the potential to reverse visual impairment by directly replacing dysfunctional RPE with healthy cells, is gathring global interest. [see references 1 and 2 for details].
Reference
- 1. Maeda T, Mandai M, Sugita S, Kime C, Takahashi M. Strategies of pluripotent stem cell-based therapy for retinal degeneration: update and challenges. Trends Mol Med. 2022 May;28(5):388-404. doi: 10.1016/j.molmed.2022.03.001. Epub 2022 Apr 1. PMID: 35370091.
- 2. Maeda T, Sugita S, Kurimoto Y, Takahashi M. Trends of Stem Cell Therapies in Age-Related Macular Degeneration. J Clin Med. 2021 Apr 20;10(8):1785. doi: 10.3390/jcm10081785. PMID: 33923985; PMCID: PMC8074076.
What is regenerative medicine?
Over the past decade(s), there have been remarkable advancements in the field of regenerative medicine, attracting global interest as a promising remedy for diseases that were previously untreatable. Particularly in Japan, spearheaded by our CEO Masayo Takahashi since 2013, there has been a series of clinical studies evaluating the use of iPS-derived cells in patients, such as neurons, cardiomyocytes, cornea, and so on. Similarly, there are 22 products, developed from somatic stem cells, now approved by the Japanese regulatory authority. They are adopted at hospitals to treat conditions like burns, cartilage damage, heart failure, and spinal cord injuries.
Retinal regenerative medicine possesses distinct advantages above other areas of regenerative medicine using iPS cells. Low production costs, smaller volume of cells required per patient, existing surgical techniques, and high-resolution imaging techniques give retinal regenerative therapy high chance of success. Thanks to accumulated clinical experiences, VCCT is well positioned to become the world-leader of regenerative medicine.
Derivation of pluripotent stem cells for regenerative therapy

What are iPS cells?
Induced pluripotent stem (iPS) cells are a type of stem cell that is reprogrammed from adult cells to return to an embryonic-like state, similar to embryonic stem cells (ES cells). This process enables these cells to acquire the capability to differentiate into nearly all types of cells in the body.
iPS cells were first reported in 2006 by a team led by Professor Shinya Yamanaka of Kyoto University, who succeeded in establishing iPS cells from mouse skin cells. Initially, Yamanaka’s team identified 24 genes believed to be key in maintaining cellular pluripotency, which is the ability of a cell to differentiate intoany type of target cell. Then, by employing a process of elimination, they discovered that only four genes were necessary to reprogram adult cells back to an embryonic-like state [Reference 1]. These genes – Oct3/4, Sox2, c-Myc, and Klf4 – are now known as the Yamanaka factors.
This revolutionary finding implied the potential to convert any cell in the body into a cell similar to pluripotent stem cells derived from an embryo, thus opening up new frontiers in regenerative medicine. The ability to create iPS cells from a patient’s own cells could reduce the risk of rejection during transplantation, paving the way for personalized medicine.
For this groundbreaking work, Dr. Yamanaka, along with Dr. John Gurdon, who had conducted pioneering research on cellular reprogramming, was awarded the 2012 Nobel Prize in Physiology or Medicine. Since then, Dr. Yamanaka has been recognized with numerous other awards for his exceptional research contributions.
Cells used in regenerative medicine (Somatic Stem, ES, and iPS cells)
Cells utilized in regenerative medicine, such as somatic stem cells, embryonic stem cells (ES cells), and induced pluripotent stem cells (iPS cells), are characterized by their self-replicating capabilities and pluripotency. ES cells are stem cells established from a cluster of cells within a blastocyst following fertilization, and they can differentiate into almost all types of cells (tissues). iPS cells were first induced from mouse fibroblasts using four transcription factors (Oct3/4, Flk1, Sox2, c-Myc) by Dr. Shinya Yamanaka of Kyoto University. In the following year, the same group succeeded in creating human iPS cells, which are now widely used for regenerative medicine, drug screening, as well as in basic research. In contrast, adult somatic stem cells exist in living tissues and are maintained in a special niche microenvironment. They are believed to be involved in tissue regeneration and capable of replacing cells lost during development and tissue damage.
Derivation of iPS cells and its differentiation
Initially, iPS cells were created from skin cells, but subsequent studies have shown that they can be generated from various other cell types. The current golden standard is the derivation from blood cells due to its process simplicity. The genes used to induce iPS cells have also been modified, and the methods used to introduce these genes have evolved from retroviruses to plasmids and Sendai viruses.
Despite the technical advancement, it is important to note that iPS cells are inherently heterogeneous and unstable. Even when generated using the same process, individual variations can lead to significant differences in gene expression levels across clones of iPS cells. Similarly, the same cell line can behave differently depending on the cell culture techniques of technicians or other environmental cues. Even when following the same protocol, the cells can change during culture and passage, highlighting the complexity of working with these cells. Over the decade of research, our scientists leveraged insights from the broader field of stem cell research to guide differentiation of these iPS cells into Retinal Pigment Epithelial (RPE) cells. We could develop a protocol that can consistently derive and isolate pure RPE cells, regardless of the source and nature of iPS cells. Once RPE cells are mature, they are known to be inherently anti-tumourigenic – historically there has been no clinical report of malignant cancer originated from RPE cells. Taken together, we believe our protocol is opt to ensure patient safety when applied for RPE cell therapy. [Reference 2, Figure 2].
Reference
- 1. Induction of pluripotent stem cells from mouse embryonic and adult fibroblast cultures by defined factors. Takahashi K, Yamanaka S. Cell. 2006 Aug 25;126(4):663-76.
- 2. Characterization of human induced pluripotent stem cell-derived retinal pigment epithelium cell sheets aiming for clinical application. Kamao H, Mandai M, Okamoto S, Sakai N, Suga A, Sugita S, Kiryu J, Takahashi M. Stem Cell Reports. 2014 Jan 23;2(2):205-18.
- 3. HLA-Matched Allogeneic iPS Cells-Derived RPE Transplantation for Macular Degeneration. Sugita S, Mandai M, Hirami Y, Takagi S, Maeda T, Fujihara M, Matsuzaki M, Yamamoto M, Iseki K, Hayashi N, Hono A, Fujino S, Koide N, Sakai N, Shibata Y, Terada M, Nishida M, Dohi H, Nomura M, Amano N, Sakaguchi H, Hara C, Maruyama K, Daimon T, Igeta M, Oda T, Shirono U, Tozaki M, Totani K, Sugiyama S, Nishida K, Kurimoto Y, Takahashi M. .J Clin Med. 2020 Jul 13;9(7):2217.
Regenerating RPE Cells to treat RPE cell dysfunction
We are teaming up with the Kobe City Eye Hospital to build upon years of their clinical experiences and continue clinical studies to evaluate the effect of iPS-derived RPE cell transplantation. In this study, we are applying our therapy to a broader population of patients with various indications involving RPE dysfunction. This will allow us to precisely understand which patient groups will benefit the most from the RPE regenerative therapy. We are also evaluating the use of our new therapy formulation “RPE strips” in comparison to previous generations, developed to enhance the safety of surgery and improve the engraftment of therapeutic cells. With the outcome of these clinical studies, VCCT will bring the therapy as early as possible to patients eagerly waiting for the new therapy.
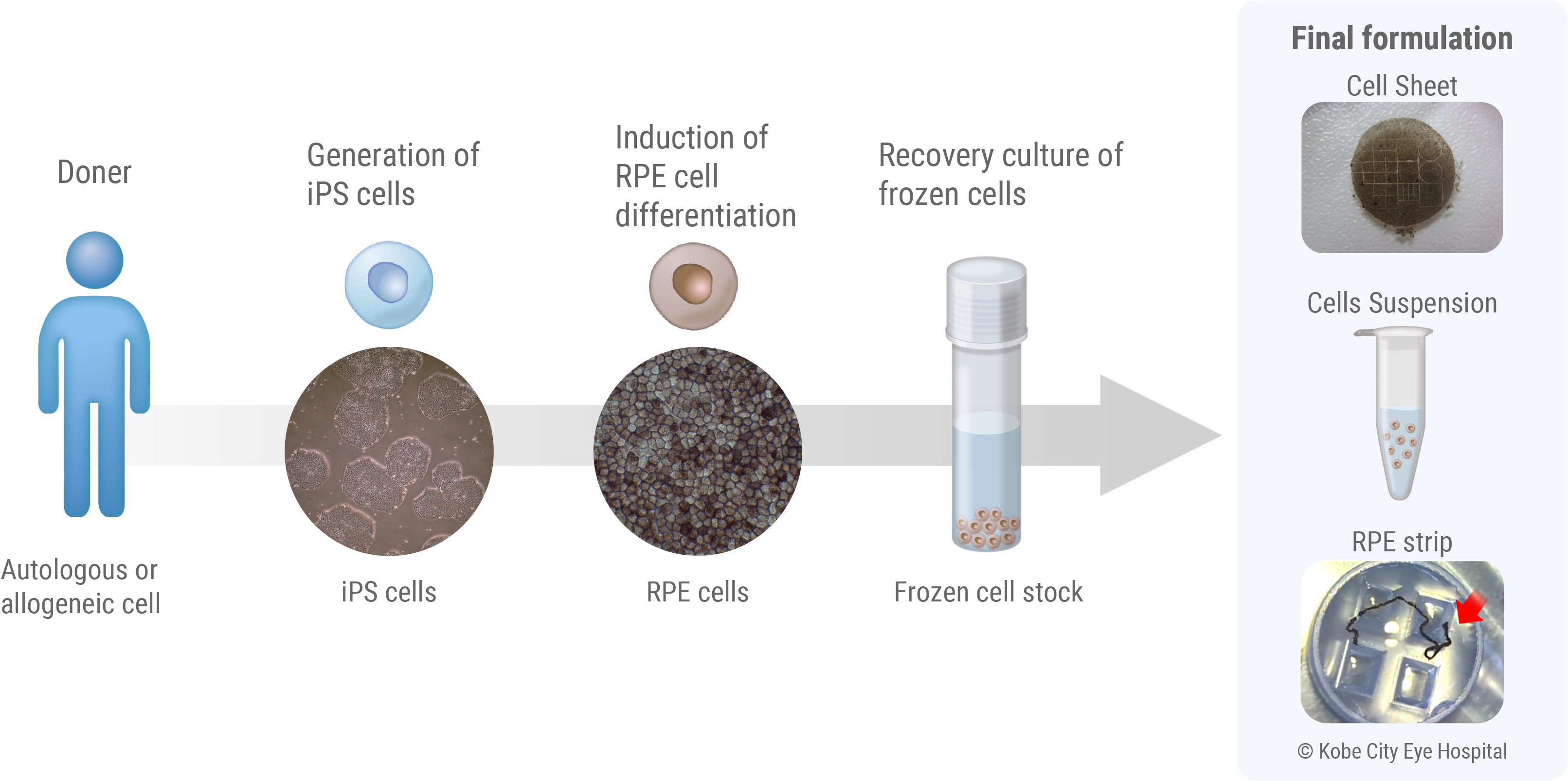
What is the Retinal Pigment Epithelium (RPE)?
The Retinal Pigment Epithelium (RPE) is a single layer of cells that is formed by the hexagonal, brown-pigmented RPE cells arranged closely together like tiles or a mosaic. It is located at the outermost part of the retina. RPE cells directly interact with and protect the photoreceptor cells, which are critical components of the retina. These cells play an essential role in maintaining our vision. Specifically, they regenerate visual pigments to sustain the light sensitivity of photoreceptors through the visual cycle, secrete nutrient molecules to alleviate the stress induced by continuous exposure to light signals, and maintain the eye’s immunological isolation by forming the retinal blood barrier between the choroid and the retina. It is known that abnormalities in RPE cells (RPE deficiency) can cause a variety of retinal degenerative diseases, including age-related macular degeneration. Unfortunately, even with the advancement of medical technology today, there is no way to heal retina cells that have degenerated or disappeared.
Autologous and Allogeneic Transplants
The iPS cells used in our regenerative medicine can be broadly divided into autologous and allogeneic (heterologous) cells. Autologous cell therapy is a treatment method in which cells collected from the patient themselves are cultured and processed, then returned to the patient. While this method can avoid risks such as immune rejection reactions and infectious diseases caused by transplanted cells, it requires cell culture and processing for each patient, resulting in substantial amount of time and cost required for the treatment per patient. Moreover, due to individual differences in cellular characteristics, there may be variations in the quality of the final product. To address these challenges, researchers are developing cost-effective methods for producing autologous iPS cells, including automating the cell manufacturing process.
On the other hand, allogeneic (heterologous) cells are collected from a chosen healthy donor (another person) for transplantation, which may have high chance of eliciting an immune rejection reaction. However, when compared to autologous cells, this approach can potentially reduce the time, cost, and quality variations associated with treatment, making it more suitable for practical use. Additionally, efforts are in progress to prepare iPS cells with specific alterations to the Human leukocyte Antigen (HLA), which is responsible for rejection reactions. These changes, made through genetic editing, aim to further enhance the feasibility and speed of practical applications.
First-ever human transplantation of iPS-derived cells
In 2014, Our CEO Masayo Takahashi, then at the RIKEN Laboratory for Retinal Regeneration, led a landmark clinical application of iPS cells. We carried out the world’s first subretinal transplantation of iPS-derived RPE cells for treating exudative AMD [Reference 1,2]. This achievement marked the earliest application of human iPS cells since their discovery in 2006. To ensure safety and efficacy in this pioneering therapy, rigorous quality control tests, including whole-genome and exome analyses, were conducted. The team managed to overcome numerous regulatory challenges, thus making a significant stride in the field.
The treatment involved procedures for neovascular removal and transplantation of iPS cell-derived RPE sheets. Throughout the process, no adverse events were observed. A year-long follow-up revealed no abnormalities. Furthermore, the grafted retinal structure was well maintained [1]. Impressively, even 7-years post-surgery, the transplanted RPE sheet remained intact without signs of tumorigenesis or abnormal cell proliferation.
The patient, a 78-year-old at the time of surgery, had previously received 13 intravitreal injections of anti-VEGF agents to suppress neovascularization due to exudative age-related macular degeneration. Remarkably, for over 7-years post-surgery, the patient required no further intravitreal injections and maintained pre-operative visual acuity.
PE cell formulation advancements
RPE cells function as a sheet-like tissue under the retina. While preparing a RPE sheet as a graft allows for accurate placement, the creation of an RPE cell sheet is time-consuming and labor-intensive. Additionally, this approach requires large incisions in the eyeball to place the sheet under the retina, making it surgically challenging. On the other hand, administering RPE cells as cell suspension allows for easier preparation, storage, and requires only a small surgical incision. However, it is challenging to ensure that precise number of cells are delivered to the transplantation site.
To overcome the disadvantages of both cell sheet and cell suspension methods, we have developed the RPE strip [2]. By culturing iPS-derived RPE cells in narrow pre-formulated grooves, we can form a strip of cell aggregate, approximately 200μm in diameter and about 2cm in length. Once this strip is placed on a culture dish, we observe RPE cells starting to grow from it the next day. As the cultivation continues, these cells expand into a sheet while expressing mature RPE markers, such as BEST1 and ZO-1.
In November 2022, we initiated clinical studies using the RPE strip, demonstrating its potential to be transplanted to targeted areas underneath the retina with minimal invasion, thereby complementing the shortcomings of both cell sheet and cell suspension methods. Furthermore, a few weeks post-transplantation, it was observed that the strip expanded into a monolayer sheet, effectively integrating into the retina.
Gene-Edited RPE Cells for Immune Evasion
One significant factor to consider in iPS cell transplantation is the presence or absence of recipient’s immune response. Our first clinical trial in 2014 employed autologous iPS cells, i.e., those derived from the patient’s own body, to minimize the risk of rejection. This method leverages the Human leukocyte antigen (HLA), a cell surface molecule instrumental in distinguishing self from non-self. The immune system eliminates cells bearing non-self HLA, ensuring the body’s protection. Therefore, in an autologous transplant, there’s no need for immunosuppressants to manage HLA-mismatch-induced rejection responses. However, this approach has its drawbacks; it requires extensive preparation time, labor, and substantial cost due to the need to establish iPS cells for each patient.
To overcome these challenges, the Center for iPS Cell Research and Application (CiRA) at Kyoto University launched the iPS Cell Stock Project for Regenerative Medicine. The project provides iPS cells from donors homozygous for the six major HLA loci, thereby reducing the likelihood of rejection in patients who share at least one pair of each of the six major HLA loci with the donor.
Our 2017 clinical trial used these HLA-matched RPE cells for transplantation. We demonstrated that such cells could be safely administered without necessitating systemic immunosuppressant use [Reference 3].
Despite the advantages of HLA-matched cells, this method is still limited to patients whose HLA type aligns with the six major HLA homozygous iPS cells provided by CiRA. As a solution, we established iPS cells using gene editing to remove part of the six major HLA loci and differentiated these into RPE cells [Reference 4]. The eye exhibits stronger immunosuppressive properties than other organs, which further lowers the risk of rejection. In vivo and in vitro validations revealed no rejection responses to these modified RPE cells. By retaining some HLA, we maintain natural immunity against tumors and resistance to viruses, combining both safety and efficacy in suppressing the rejection response for intraocular transplantation.
Reference
- 1. Mandai M, Watanabe A et al. N Engl J Med 2017
- 2. Takagi S, Mandai M et al. Opthalmol Retina 2019
- 3. Sugita S, Mandai M et al. J Clin Med 2020
- 4. Ishida et al., in preparation
Regeneration of photoreceptor cells
Photoreceptors are specialized cells responsible for light perception, and hence its loss is the ultimate cause of vision loss in retinal disorders. Its degeneration attributes to either mutations in genes related to development, function, or structural integrity of photoreceptor cells, or dysfunctional RPE cells which are essential for keeping photoreceptor cells functional. Once photoreceptor cells are lost, it cannot be regenerated and there is no effective treatment available today. VCCT is developing a regenerative medicine solution to this problem. We use allogeneic iPS cells and convert them into retinal organoids. Retinal organoids are reminiscent to immature retina and, once transplanted into patients, have the potential to regenerate photoreceptor cells. These photoreceptor cells form new synaptic connections with surrounding neurons, thus re-creating a new light signalling pathway within otherwise visually impaired patients. We use allogenic iPS cells as a starting material because our previous research has shown that retinal organoids are minimally immunogenic, meaning there is a limited chance of grafts getting rejected by the host. In collaboration with the Kobe City Eye Hospital, we are continuing clinical studies to affirm the safety and efficacy of this new therapy.
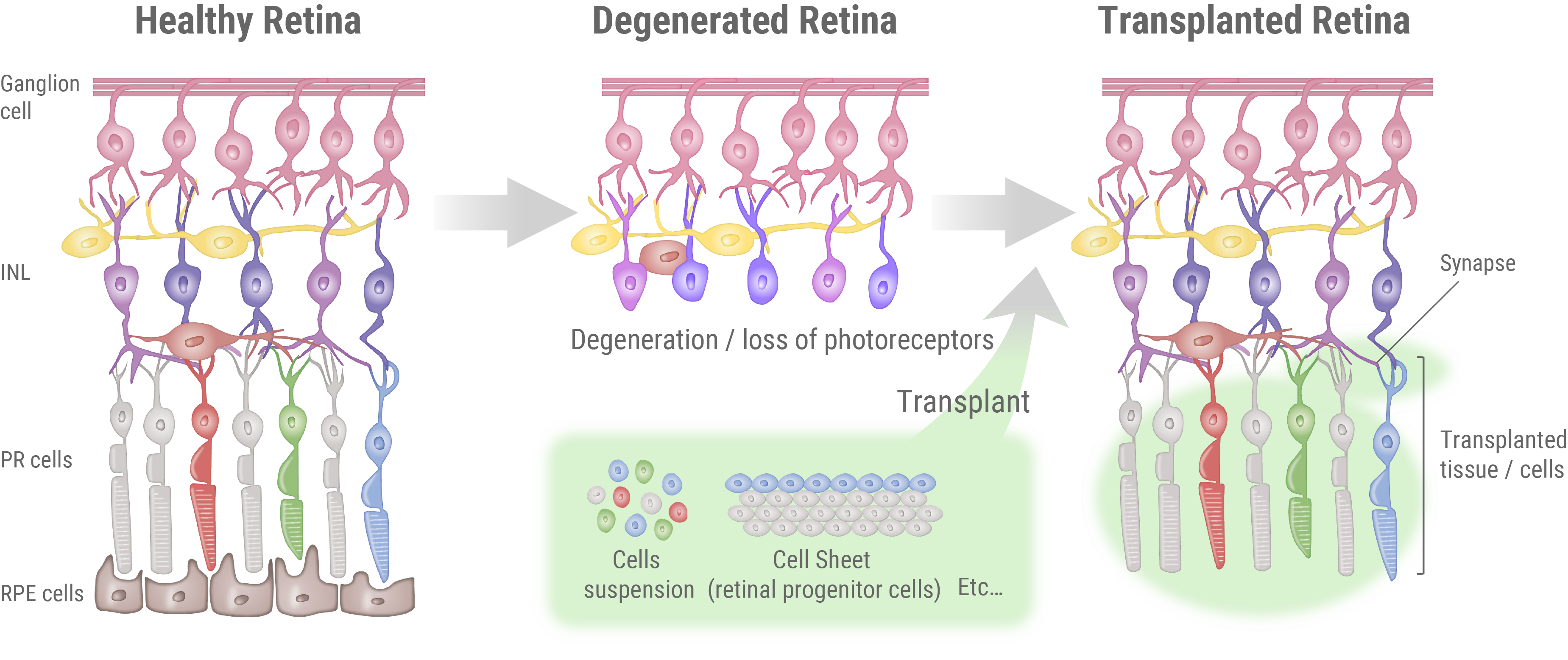
What are Photoreceptor cells?
Photoreceptor cells are specialized nerve cells that act as light receptors, converting light into chemical signals and transmitting this visual information to secondary neurons, such as bipolar cells. These cells are located in the farthest layer of the retinal tissue within the eye, closest to the sclera (or the white part of the eye). They fall into two categories: rod cells, which facilitate vision in dim light, and cone cells, responsible for sharp vision and color perception. Cone cells are further divided into blue, green, and red types, based on their sensitivity to different light wavelengths, playing a crucial role in color vision. A distinguishing feature of photoreceptor cells is an intricate structure known as the outer segment, which efficiently captures light. This structure is densely packed with light-receptor molecules called opsins.
Development and Clinical Applications of Retinal Organoids
Retinal organoids are 3D constructs that replicate the structure of in vivo retinas. They can be produced in vitro from ES or iPS cells, facilitated by specific soluble factors that induce differentiation. Interestingly, these organoids mimic the natural progression of retinal development, establishing a complex three-layered structure comprising of the outer nuclear layer, inner nuclear layer, and ganglion cell layer. During this process, all cell types, including photoreceptor cells, differentiate from retinal progenitor cells.
Retinal organoids can be produced utilizing different soluble factors. A popular strategy involves differentiating ES/iPS cells to the retina via the neural ectoderm. Our research employs a method utilizing Bone Morphogenetic Protein 4 (BMP4), a part of the Transforming Growth Factor-beta (TGFβ) family. We use the Serum-free Floating culture of Embryoid Body-like aggregates with quick reaggregation (SFEBq) technique, where ES/iPS cell aggregates are suspended, and BMP4 is applied to induce differentiation towards retinal cells. This approach has enabled the production of high-quality retinal organoids, which was instrumental in the world’s first transplantation of an iPS cell-derived retinal sheet into a patient with RP in 2020 at the Kobe City Eye Hospital. Our ongoing efforts are centered around using this technique to develop larger retinal organoids for transplantation.
Furthermore, retinal organoids differentiated from iPS cells of patients with retinal degenerative diseases offer potential tools for pathology analysis and for developing treatments to halt disease progression. We aim to leverage retinal organoids as transplant tissues, targeting the restoration of lost vision and visual fields.
Effectuality of photoreceptor cell transplantation
Our transplantation process primarily utilizes retinal and photoreceptor precursor cells, which undergo post-transplantation maturation. This process establishes a light signal transmission pathway. Notably, after maturation electron microscopy reveals the formation of specialized light detection compartments, known as rod outer segments.Simultaneously, the cells express essential functional molecules intrinsic to photoreceptor cells, such as opsin and transducin. Our primate-based transplant trials further demonstrated the robust integration and enduring stability of transplanted photoreceptor cells, confirmed up to two years post-transplantation.
For the restoration of visual function, it is essential that transplanted photoreceptor cells are not only responsive to light stimulation but also capable of transmitting light information to downstream secondary neurons. This involves the formation of ribbon synapses, a specialized structure that facilitates the transmission of graded light signals from photoreceptor cells to second-order neurons, such as bipolar cells. This process is critical for conveying light information. Beyond the evidence from immunohistochemistry, we have utilized electrophysiological techniques to demonstrate the light signal transmission capability of transplanted photoreceptors. By transplanting sheets derived from human retinal organoids into rats with immunodeficient retinal degeneration, we recorded the light response of the host retina using a multi-electrode array (MEA) system. These findings indicate that transplanted retinas can respond to light and transmit information to host ganglion cells.
Behavioral experiments were meticulously carried out to evaluate restoration of vision. In one experiment, retinal organoids derived from mouse iPS cells were transplanted into mice with end-stage photoreceptor degeneration. The transplant recipients exhibited a response to light in shuttle avoidance tests, reflecting a successful integration of the transplanted photoreceptor cells and their physiological response to light. In a separate study, human retinal organoid-derived sheet transplants were applied to monkeys with artificially induced photoreceptor degeneration. The results from visually-guided saccades (VGS) tests suggested noticeable improvements in the visual field, further affirming the potential of our transplant approach in restoring light-induced behavior.
Prospects for Next Generation Retinal Transplantation
At present, our transplantation technique involves the use of retinal sheets derived from iPS cells. During transplantation, these sheets are primarily composed of retinal and photoreceptor precursor cells. Post-transplantation, these cells mature into photoreceptors and differentiate into secondary neurons such as bipolar and horizontal cells, as well as Müller glial cells. Our method of transplanting retinal sheets, offers consistent long-term integration and stability of the transplanted photoreceptor cells, a feature that distinguishes it from other transplantation methods using purified photoreceptor cells in suspension.
However, there is a potential challenge that photoreceptor cells cannot establish synaptic connections with host secondary neurons, hindered by presence of bystander cells. In response to this, we are improving our strategies to maximize therapeutic outcomes with gene editing. By modifying the differentiation and maturation trajectory of the transplanted retina, we aim to optimize the efficiency of synaptic connections between the host and transplanted retina.
Cell Manufacturing Technology
Manufacturing iPS-derived cells for regenerative medicine is challenging. This is because, despite the use of standardized protocols, the outcome or yield from production varies batch-to-batch significantly depending on the skills of technicians. For example, even small changes in the speed of adding culturing medium to iPS cells have a profound effect on their health and capacity to differentiate into other cell types. As such, every clinical trial currently undergoing globally relies on the implicit knowledge and expertise of seasoned technicians. At VCCT, we are solving this issue with the use of humanoid robots, Maholo. We have started to transition our manufacturing process to robotics-based production, and successfully improved its reproducibility.
Technique handover from Human to Robots (&AI)
In collaboration with the Robotic Biology Institute Inc., developers of the general-purpose humanoid robot LabDroid “Maholo”, and Epistra Inc., VCCT has established the next-generation cell processing system using robotics and AI. Maholo is now set up to carry out any procedures using its two arms to handle identical apparatus and tools as a human [Figure 1]. AS reported by RIKEN, this system can successfully cultivate iPS cells under 143 conditions, chosen from 200 million potential parameter combinations, over 111 days [Reference 2,3]. The results demonstrated a production of iPS-RPE that was 88% more efficient compared to pre-optimized cultures, as assessed by pigment appearance scores (RPE cell ratios). Additionally, we have developed a system in which Maholo autonomously passaged cells at the predicted time when the cell culture would become 80% confluent [Reference 3]. These experiments demonstrated high potential for such robots and AI system to be able to autonomously conduct biological experiments.
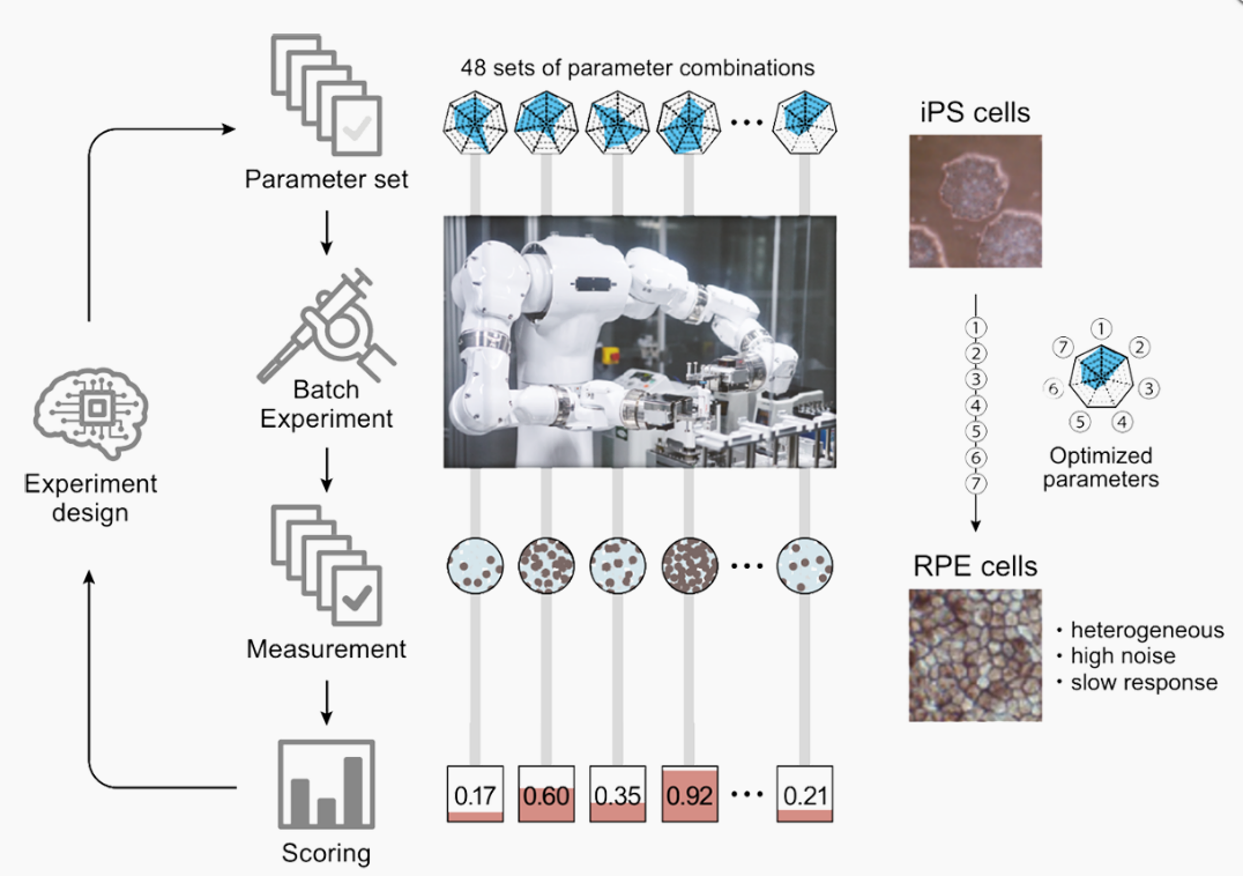
Reference: Kanda et al., 2022, elife
Cell Manufacturing through Robotics and AI
Our company is propelling the techniques developed in the realm of basic research, towards automated cell manufacturing by the use of robotics and AI. We are striving to devise processes that consistently generate high-quality cells suitable for clinical use. We’ve already achieved approval from the Committee for Designated Regenerative Medicine and the Health Sciences Council for clinical trials at the Kobe City Eye Hospital, where Maholo assists in the iPS-RPE production. This innovative incorporation of robotics in clinical cell production has led to a more stable manufacturing process. By optimizing cell culturing techniques without altering human culture and protocols, we’ve been able to seamlessly transition this approach into clinical cell production.
Additionally, our cell culture processing facility, known as the Facility for iPS-derived retinal stem cell therapy (FiRst, approximately 357 m²), received a “Special Cell Processing Product Manufacturing License” from the Kinki Bureau of Health and Welfare of the Ministry of Health, Labour and Welfare on October 20, 2022. FiRst is designed with two independent cell processing rooms, allowing for ongoing manufacturing in one room while methodological enhancement and experimentation in the other, thereby ensuring smooth automation implementation. It is set to carry out cell manufacturing for future clinical trials.
Reference
- 1. Robotic crowd biology with Maholo LabDroids. Yachie N; Robotic Biology Consortium; Natsume T. Nat Biotechnol. 2017 Apr 11;35(4):310-312. doi: 10.1038/nbt.3758.1
- 2. Robotic search for optimal cell culture in regenerative medicine. Kanda GN, Tsuzuki T, Terada M, Sakai N, Motozawa N, Masuda T, Nishida M, Watanabe CT, Higashi T, Horiguchi SA, Kudo T, Kamei M, Sunagawa GA, Matsukuma K, Sakurada T, Ozawa Y, Takahashi M, Takahashi K, Natsume T. Elife. 2022 Jun 28;11:e77007. doi: 10.7554/eLife.77007.
- 3. A Variable Scheduling Maintenance Culture Platform for Mammalian Cells. Ochiai K, Motozawa N, Terada M, Horinouchi T, Masuda T, Kudo T, Kamei M, Tsujikawa A, Matsukuma K, Natsume T, Kanda GN, Takahashi M, Takahashi K. SLAS Technol. 2021 Apr;26(2):209-217. doi: 10.1177/2472630320972109.